Abstract
The endocytic network comprises a vast and intricate system of membrane-delimited cell entry and cargo sorting routes running between biochemically and functionally distinct intracellular compartments. The endocytic network caters to the organization and redistribution of diverse subcellular components, and mediates appropriate shuttling and processing of materials acquired from neighboring cells or the extracellular milieu. Such trafficking logistics, despite their importance, represent only one facet of endocytic function. The endocytic network also plays a key role in organizing, mediating, and regulating cellular signal transduction events. Conversely, cellular signaling processes tightly control the endocytic pathway at different steps. The present article provides a perspective on the intimate relationships that exist between particular endocytic and cellular signaling processes in mammalian cells, within the context of understanding the impact of this nexus on integrated physiology.
Bạn đang xem: Endocytosis là gì
Molecular mechanisms governing the remarkable diversity of endocytic routes and trafficking steps are described elsewhere in the literature (see Bissig and Gruenberg 2013; Henne et al. 2013; Burd and Cullen 2014; Gautreau et al. 2014; Kirchhausen et al. 2014; Mayor et al. 2014; Merrifield and Kaksonen 2014; Piper et al. 2014). Moreover, these have been the focus of many studies in the last 30 years, and the topic has been covered by many excellent reviews, making it unnecessary for us to dwell on this aspect any further here (see, for instance, Howes et al. 2010; McMahon and Boucrot 2011; Sandvig et al. 2011; Parton and del Pozo 2013). Herein, we will instead concentrate our attention on how cellular regulatory mechanisms control endocytosis, as well as on how endocytic events impinge on cell functions. Emphasis will be placed, although not exclusively, on studies that analyze cellular networks using holistic approaches and in vivo analysis. Our aim is to give the reader a flavor of the deep embedding of endocytic processes within cellular programs, a concept we refer to as the endocytic matrix (Scita and Di Fiore 2010).
CELLULAR PROGRAMS REGULATING THE ENDOCYTIC PATHWAY: HIGH-THROUGHPUT STUDIES AND IN SILICO ANALYSIS OF CELLULAR REGULATORY NETWORKS
If we reflect on the pervasiveness of the endocytic network throughout the cell, we must inevitably infer that any perturbations in this system will likely have unpredictable and critical effects on cellular homeostasis (Sigismund et al. 2012). High-throughput studies of endocytic pathways have helped to characterize this complex regulatory network, paving the way for us to finally understand in molecular terms the true extent of the impact of endocytosis on cellular homeostasis, and the reciprocal modulation of endocytosis by signaling and metabolic pathways (Pelkmans et al. 2005; Snijder et al. 2009, 2012; Collet al. 2010; Lupberger et al. 2011; Mercer et al. 2012). For example, recent work has shown that different signaling events or stress responses can cause specific remodeling of endocytic compartments through the direct or indirect activation of defined endocytic pathways. Among these compartments, endosomes have emerged as critical endocytic regulatory “hubs,” in which different signals converge and signaling outcome is decided. It is here that critical decisions are made as to whether cargos will be recycled to the plasma membrane (PM), retrotransported to the Golgi, or further trafficked to late endosomes for degradation in lysosomes; in addition, endosomes actively participate to the process through which signals are deconvoluted and rendered to the cell in an understandable form (Spang 2009; Hsu and Prekeris 2010; Jovic et al. 2010).
Endosome trafficking and fusion are regulated by a specific set of molecules, including but not limited to Rab GTPases and SNARE proteins (Brocker et al. 2010; Huotari and Helenius 2011; Jean and Kiger 2012). Recently, in vitro reconstitution of endosome fusion events was achieved using a subset of 17 recombinant human proteins, including RAB5, Rab5 effectors, and SNAREs (Ohya et al. 2009). RAB proteins are not only required for fusion events but they also determine the functional organization of different endosomal compartments, by flagging distinct RAB-containing membrane subdomains both in early and late endosomes (excellently reviewed by Zerial and McBride 2001; Stenmark 2009; Huotari and Helenius 2011; Jean and Kiger 2012). RAB5 and RAB7 specify early and late endosomes, respectively, and the early-to-late endosome transition entails the replacement of RAB5 with RAB7 (Chavrier et al. 1990; Rink et al. 2005). The switch from RAB5-positive to RAB7-positive endosomes (“RAB conversion”) is achieved via the progressive accumulation of RAB7 at the endosomal membrane, coupled with the parallel removal of RAB5 (Rink et al. 2005; Wandinger-Ness and Zerial 2014), a process conserved throughout evolution (Poteryaev et al. 2010). A similar “maturation mechanism” has been reported for a subset of APPL-endosomes (Zoncu et al. 2009). In a similar vein, Julie Donaldson”s group showed that newly formed macropinosomes can be converted directly into early endosomes, through the progressive loss of PIP2 from the vesicles, concomitant to Rab5 recruitment and PIP3 enrichment (Porat-Shliom et al. 2008).
The emerging picture is that of endosomes as extremely dynamic entities. This immediately asks the question of how endosomal dynamics are controlled to respond to changing physiological needs. In the remainder of this section we will review recent advances on how signaling processes and transcriptional programs regulate the endocytic pathway.
Plasticity of the Endocytic Pathway and Control by Signaling Processes
Endosomal maturation is a tightly regulated process that controls the progressive concentration of specific cargoes at endosomal stations. For example, degradative ligands such as LDL (low-density lipoproteins) become progressively more concentrated in a decreasing number of gradually expanding endosomes migrating from the cell periphery to the center, concomitantly with the replacement of RAB5 by RAB7 (Rink et al. 2005). Importantly, growth factors can influence RAB conversion. Indeed, treatment with EGF (epidermal growth factor) slows down LDL transport by activating RAB5 and delaying the RAB5-to-7 conversion (Rink et al. 2005). The heterotrimeric GTP protein (G protein) Gs, a classical mediator of seven-transmembrane or G-protein-coupled receptor (GPCR) signaling, associates with early endosomes and promotes RAB5-to-7 conversion when in its “inactive” (GDP-bound) state (Beas et al. 2012). A similar mode of regulation, in which signaling exerts control over endocytic dynamics, was observed in multivesicular bodies (MVBs). Indeed, EGF stimulation controls both MVB biogenesis, by increasing the number of MVBs per unit of cytoplasm, and inward vesiculation, by increasing the number of intraluminal vesicles (ILVs) per MVB (White et al. 2006).
System-wide analysis of the endocytic pathway further reinforced the idea that the endosomal compartment is dynamically regulated in response to cellular needs (Collet al. 2010). Indeed, cells tightly couple the number of EGF-positive endosomes, their size, and intracellular location to cargo concentration in the endosome (Collet al. 2010). This precise regulation is expected to impact on endosomal signaling, controlling both quality and strength of signaling outputs. In the same study, it emerged that the regulation of endocytosis by signaling is exerted also at the PM. For instance, various metabolic signaling pathways, such as mTOR, integrin, TGF-βR, Notch, and Wnt, significantly alter EGF and/or TF endocytosis at early steps (Collet al. 2010). These signals might act either directly on the endocytic machinery or through regulatory feedback loops tightly controlling PM receptor level and availability.
GPCRs also illustrate plasticity of the endocytic pathway at the early stages of the route. Ligand-induced activation of β-adrenergic receptors promotes their clustering in clathrin-coated pits that effect homeostatic control of adrenergic signaling. Receptors present in these coated pits, through interaction of the cytoplasmic tail with proteins associated with the cortical actin cytoskeleton, slow endocytosis of the receptor-containing coated pits (Puthenveedu and von Zastrow 2006). Because adrenergic receptors signal via distinct “waves” of effector activation occurring sequentially from the PM and endosome limiting membrane, such control of early endocytic events by receptors can precisely sculpt the spatiotemporal profile of receptor-elicited cellular signaling (Lohse and Calebiro 2013). Opioid neuropeptide receptors also locally control-coated pit dynamics, and the delay imposed by opioid receptors is effectively “released” by ubiquitination of the same cytoplasmic residues that control the later sorting of receptors within MVBs (Henry et al. 2012). Accordingly, local control of coated pits by opioid receptors reveals an additional “checkpoint” function of cargo control that coordinates the initial endocytosis of receptors with later trafficking events. These studies reinforce the idea that endocytosis cannot be portrayed as a simple transport system from one static compartment to another. Rather, endocytosis is dynamically regulated and rearranged depending on the type and intensity of signaling, and the multiplicity of cargoes engaged.
The plasticity of the endocytic system to environmental cues further emerged through another system-wide approach that investigated context-dependent population phenotypes in endocytosis, using viruses as tools to follow endocytic pathways (Snijder et al. 2009, 2012). These studies showed that clathrin-mediated endocytosis was mostly active in densely populated cell cultures, whereas clathrin-independent pathways (measured as SV40 virus infection) were more efficient in sparsely populated cell cultures. This latter response is achieved through the activation of focal adhesion kinase (FAK) and the consequent modulation of surface levels of the sphingolipid GM1, the receptor for SV40-dependent host cell attachment and entry (Snijder et al. 2009). Thus, these studies revealed that local cell density regulates endocytic pathways.
Transcriptional Programs Regulating the Endocytic Pathway
An emerging body of evidence is highlighting the existence of highly coordinated transcriptional programs in control of endocytosis (Fig. 1) (also see Settembre and Ballabio 2014). Recent studies have exploited in silico analysis to screen publicly available microarray data for lysosomal gene expression status (Sardiello et al. 2009; Settembre et al. 2013b). The underlying idea was that genes belonging to the same pathway/organelle (the lysosome in this case) should be coexpressed, regulated by common factors, and able to respond to the same signaling and environmental cues. Indeed, it was found that lysosomal genes have a significant tendency to be coexpressed in different tissues and cell types in response to a variety of stimuli and growing conditions (Sardiello et al. 2009). Importantly, a palindromic 10 base site was identified in the promoter region of these genes, a sequence that resembles the so-called “E box,” the target site for basic-helix-loop-helix (bHLH) transcription factors. A new network, called CLEAR (coordinated lysosomal expression and regulation), was thus identified in its entirety through an in silico bioinformatics analysis. Further experiments in the wet-laboratory identified the transcription factor TFEB as a master regulator of the CLEAR network. TFEB normally binds to the promoter region of lysosomal genes and positively regulates their expression (Sardiello et al. 2009). Under specific conditions, such as when lysosomal dysfunction occurs in lysosomal storage disorders (LSDs), TFEB translocates from the cytoplasm to the nucleus and mediates the coordinated transcriptional activation of lysosomal genes. This stimulates the expression of lysosome biogenesis and function, thus facilitating the clearance of nondegraded molecules (Sardiello et al. 2009). These observations illustrate how lysosomal function is tightly and specifically modulated in response to cellular needs (a feature dubbed “lysosomal adaptation”).
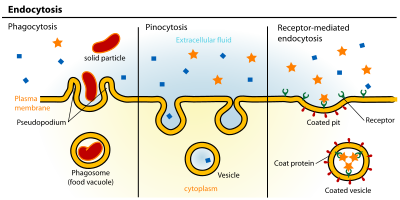
Figure 1.
Transcriptional programs controlling endocytosis. Lysosomal stress (A) and/or starvation (B) cause nuclear translocation of the TFEB transcription factor, which binds to the E-box sequence on promoters and induces transcription of a cluster of genes (the “CLEAR network”) involved in lysosomal biogenesis/function and autophagy genes, promoting cellular clearance of nondegraded molecules. (C) Activation of HIF1 during hypoxia causes the inhibition of the RABAPTIN-5 gene transcription, inhibition of Rab5 GTP-loading and, consequently, endosomal retention of the EGFR, eventually leading to sustained EGFR signaling from the endosomal station and tumor progression. (D) On different types of cellular stresses, p53 translocates into the nucleus and activates the transcription of genes that play roles at different stations of the endocytic pathway: autophagy genes (e.g., lysosome/autophagosome components), genes involved in exosome release from MVBs (e.g., ESCRT components), and the CAV-1 gene, which stimulates caveolar endocytosis. (E) TP53 mutations found in human cancers exert part of their oncogenic potential through the P63-dependent (transcriptional-dependent) stimulation of RCP-mediated recycling of integrin-EGFR complexes, leading to induced migration and metastasis. The molecular mechanism for this remains unclear.
The CLEAR network contains not only lysosomal proteins but also autophagy substrates (Palmieri et al. 2011). Indeed, a response similar to that observed in LSDs occurs during cell adaptation to starvation, when cells switch to an energy-saving mode of “waste not, want not” by recycling their cellular components to help cater for their metabolic needs (Lieberman et al. 2012). This observation led to the discovery that TFEB also regulates the autophagy pathway via the CLEAR network (Settembre et al. 2011, 2013a). Thus, cells can use the same transcriptional program to regulate distinct, yet mechanistically related, endomembrane-based processes. Thanks to this pioneering work, the concept of plasticity and adaptation of the endocytic system to cellular needs has emerged more clearly than ever, and great attention is now given to the study of transcriptional programs controlling endocytic genes and pathways. Indeed, the CLEAR network is just one example of how the stress-induced transcriptional regulation of endocytic proteins can be used to regulate specific cellular responses; other examples are described below.
Under hypoxia conditions, a transcriptional regulatory loop regulates RAB5 activity. Indeed, the hypoxia-inducible factor HIF1α was shown to inhibit the transcription of RABAPTIN-5 gene, a critical RAB5 effector (Wang et al. 2009), thus impairing RAB5-mediated early endosome fusion and delaying the endocytic pathway. As a consequence, the resident time of activated EGFR in endosomes is prolonged and signaling is sustained leading to cell proliferation and survival (Wang et al. 2009). In agreement with this mechanism, tumor hypoxia and HIF1α overexpression generally correlate with an aggressive phenotype and poor patient prognosis (Zhong et al. 1999; Wang et al. 2009). Importantly, primary kidney and breast tumors with strong hypoxic signatures show significantly lower expression of rabaptin-5 mRNA and protein (Wang et al. 2009).
Stress signals activating TP53-dependent transcriptional programs have also been shown to affect the endocytic compartment. Indeed, TP53 regulates genes involved in exosome production, as well as lysosomal membrane proteins required for the induction of the autophagy pathway (Yu et al. 2009b). In addition, TP53 transcriptionally regulates caveolin-1, a regulator of caveolar function. As a consequence of TP53 activation, caveolin-1 and EGFR are reported to be internalized concurrently from the PM, and directed to the MVB compartment for degradation (Yu et al. 2009b). In this way, the TP53 program, by regulating the endocytic pathway, could contribute to suppressing cell growth and division in response to stress. Furthermore, it appears that the oncogenic potential of TP53 point mutants found in human cancer may partly lie in their regulatory role within the endocytic pathway (Muller et al. 2009), because it has recently been shown that these mutants not only lose tumor suppression activity, but they also promote invasion and metastasis by inducing RCP (RAB-coupling protein)-mediated recycling of integrins and EGFR, thus sustaining AKT signaling and inducing cell migration (Muller et al. 2009). The mechanism involved is not yet completely understood, but it possibly relies on the inhibition of p63 (a TP53 family member) transcriptional activity.
Xem thêm: Bit Ly Là Gì – Cách Rút Gọn Link Bit
UNDERSTANDING THE IMPACT OF ENDOCYTOSIS ON CELL PHYSIOLOGY: STUDIES AT THE LEVEL OF REDUCED PHYSIOLOGICAL SYSTEMS
One approach to exploring the physiological impact of endocytosis is through examining, in ex vivo or in vitro preparations, particular cellular processes that have well-established parallels in vivo. A few examples are briefly described below, based on studies of cardiovascular and nervous system regulation in mammals.
Endocytosis in Sympathetic Control of Cardiac Function
The sympathetic nervous system exerts physiological control of cardiac contraction strength and rate by locally releasing catecholamines that activate β1- and β2-adrenergic receptors, two closely related GPCRs that are coexpressed on the surface of cardiac myocytes (Fig. 2). β2-Adrenergic receptors (B2ARs) have the interesting ability to couple either to Gs or Gi, distinct heterotrimeric G protein mediators that exert opposite effects on cardiac contraction rate. On initial activation, when most receptors are located in the PM, the heterotrimeric G protein Gs is activated and cardiac muscle contraction is accelerated. Activated receptors undergo regulated endocytosis followed by recycling to the PM, which is associated with a “switch” of receptor coupling to the distinct G protein Gi, and a slowing of cardiac muscle contraction. The endocytic pathway is proposed to mediate this switch by removing receptors from a region of relative Gs enrichment and inserting recycled receptors into a region of relative Gi enrichment (Xiang and Kobilka 2003). β-Adrenergic receptor recycling is dependent on a carboxy-terminal PDZ motif and RAB4, and mediated by receptor engagement with a complex sorting machinery associated with the limiting membrane that includes sorting nexin 27, a WASH-Arp2/3 actin nucleation complex, and the retromer complex (Lauffer et al. 2010; Puthenveedu et al. 2010; Temkin et al. 2011; Steinberg et al. 2013). Supporting validity of these concepts to higher level ex vivo and in vivo function, disrupting PDZ motif-linked (through sorting nexin 27) engagement of receptors with this machinery selectively abrogates the Gi component of signaling in isolated cardiac myocytes (Xiang and Kobilka 2003). Moreover, inhibiting RAB4 activity in cardiac muscle cells in vivo (using transgenic mice) disrupts catecholamine control of cardiac contractility and leads to heart failure (Odley et al. 2004).
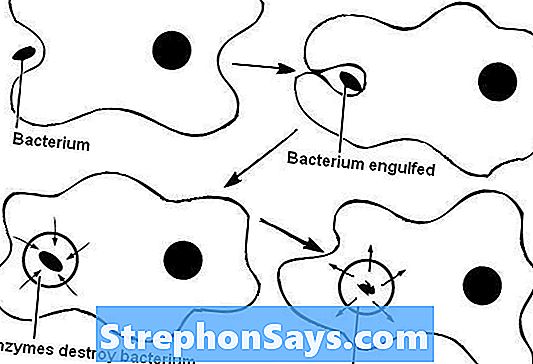
Figure 2.
Endocytosis modifies GPCR signaling and vice versa. (A) Activation of the β2-adrenergic receptor by catecholamine stimulates adenylyl cyclase through coupling to the heterotrimeric G protein Gs, thereby increasing the cAMP concentration in the cytoplasm. Receptor activation also initiates a process of regulated endocytosis of receptors, mediated by clathrin-coated pits, delivering activated receptors to early endosomes. (B,C) In endosomes receptors are thought to activate noncanonical signaling through the scaffold protein β-arrestin β-Arr, leading to activation of MAP kinases (B), and initiate a second “wave” of Gs-mediated activation of adenylyl cyclase that further increases cytoplasmic cAMP concentration (C). Receptors recycle to the PM by engaging a multiprotein sorting machinery, including sorting nexin 27, and the retromer complex (depicted by SNX-RM in the diagram). (D) On return to the PM, receptors couple to Gi, reducing adenylyl cyclase activity and decreasing cytoplasmic cAMP concentration. In cardiac muscle cells, this series of events causes an acute acceleration (Gs response) followed by deceleration (Gi response) of cardiac contraction (indicated by “+” and “−” arrows in figure). The GPCR-G protein system conversely regulates the endocytic process in at least two ways: (E) β-Adrenergic receptors locally prolong the surface lifetime of clathrin-coated pits that contain them, and (F) Gs acts in the early endosome membrane to promote endosome maturation through RAB5-to-7 conversion.
Endocytosis in the Control of Synaptic Neurotransmission
Fast synaptic transmission occurs at central nervous system (CNS) synapses by exocytic release of neurotransmitter from the presynaptic terminal that, in turn, activates ligand-gated ion channels across the synaptic cleft in the postsynaptic PM. In mammals, fast excitatory neurotransmission is mediated primarily by presynaptic release of glutamate and by subsequent activation of postsynaptic glutamate-gated cation channels, resulting in depolarization of the postsynaptic neuron. In some CNS synapses, such as the extensively studied CA3-CA1 synapse in the hippocampus, the efficacy or strength of excitatory neurotransmission is subject to exquisite regulation through endocytosis and recycling of critical postsynaptic signaling receptors that reduce or increase, respectively, synaptic strength and thereby impact learning (Luscher et al. 1999; Kessels and Malinow 2009). At the CA3-CA1 synapse, the major receptor species subject to rapid regulation by endocytic trafficking is an AMPA-type glutamate-activated cation channel (AMPA receptor), which appears to engage a similar sorting nexin 27 dependent sorting machinery as that engaged by β-adrenergic receptors (Temkin et al. 2011; Steinberg et al. 2013; Wang et al. 2013). AMPA receptor engagement of this sorting machinery supports efficient recycling of internalized AMPARs and is essential for sustaining or increasing synaptic strength. Disrupting this sorting machinery, conversely, results in inappropriately weak synaptic responses and failure of synapses to potentiate their responsiveness to salient stimuli. Such a disruption of AMPAR recycling was proposed recently to underlie cognitive defects observed in a mouse model of Trisomy 21 or Down syndrome (Wang et al. 2013).
Endocytosis in the Control of Integrated Neuronal Excitability
In addition to fast synaptic transmission through ligand-gated ion channels, information flow in the nervous system is also affected by slower neuromodulatory processes that affect the electrical excitability of neurons, thus determining how much neurotransmitter will be released presynaptically or how likely it is that activation of a given number of ligand-gated ion channels in the postsynaptic cell will trigger an action potential. Much of this slower neuromodulation is mediated by GPCRs, which are typically expressed on the surface of axons and dendrites outside of, or adjacent to, synaptic specializations. Many of these receptors are regulated by activation-induced endocytosis, and for GPCRs such as the D1-type dopamine receptor and µ- and δ- type opioid receptors, this has been clearly shown in vivo (Sternini et al. 1996; Dumartin et al. 1998; Keith et al. 1998; Pradhan et al. 2009). The functional significance of this trafficking remains poorly understood, but the evidence available so far suggests that the consequences may be quite varied. Opioid receptor endocytosis reduces tissue opioid responsiveness (Pradhan et al. 2009) or recovery of tissue responsiveness (Quillinan et al. 2011) after prolonged exposure, and D1 dopamine receptor endocytosis increases the acute signaling response, apparently through receptor-mediated initiation of G protein-linked signaling from endosomes in addition to the PM (Kotowski et al. 2011; see also Morgan et al. 2013 and Cosker and Segal 2014 regarding endocytosis and neuronal function).
Xem thêm: Adrenergic Là Gì – Giải Thích Một Số Thuật Ngữ
UNDERSTANDING THE IMPACT OF ENDOCYTOSIS ON CELL PHYSIOLOGY: INTEGRATION AT THE LEVEL OF TISSUE AND ORGAN HOMEOSTASIS
The examples described in the previous paragraph highlight how, through the analysis of physiologically relevant systems in vitro, we are developing a stronger sense of how endocytosis impacts on cellular functions and their regulation. A higher level of understanding entails the analysis of how endocytosis impacts the establishment and maintenance of intact tissue and organ homeostasis and on their function. Not surprisingly, model organisms have paved the way for this kind of analysis during development. More recently, in vivo studies in adult mammals have also helped to understand how homeostasis and functionality are maintained by endocytic compartmentalization at the organ level. Here following, we will provide some recent examples of in vivo studies that highlight the importance of the endocytic process in the regulation of tissue differentiation and homeostasis, by concentrating on how endocytosis affects intercellular communication and polarity.
Endocytosis, Spatial Segregation of Signals, and Intercellular Communication
Endocytosis can restrict signaling spatially and temporally within the cell, as shown in vitro for different RTKs (for a review see Sorkin and von Zastrow 2009; Scita and Di Fiore 2010) and, more recently, as shown unequivocally for GPCRs (Irannejad et al. 2013). This level of regulation controls numerous cell autonomous functions. However, it also impacts profoundly on intercellular communication (excellently reviewed recently in Shilo and Schejter 2011). Many developmental functions are commanded by morphogen gradients, irradiating from signal-sending cells, which differentially instruct target cells, depending on the position of the latter with respect to the gradient. Endocytosis of morphogens by target cells shapes the gradient, as shown for several signaling systems (see, for instance, Kicheva et al. 2007; Yu et al. 2009a; Gonzalez-Gaitan and Jülicher 2014). However, there is now evidence that endocytosis is also critical in the interpretation of the gradient by target cells. By in vivo fluorescence correlation spectroscopy studies, Nowak and coworkers were able to monitor FGF receptor activation and intracellular distribution of the active receptor in zebrafish embryos (Nowak et al. 2011). These experiments revealed that the balance between endosomal versus PM signaling is crucial to interpret morphogen gradients in vivo (FGF8 in this case). Indeed, during zebrafish development, morphogen gradients provide positional information to individual cells within a tissue, thereby determining their fate. Importantly, expression of a Cbl dominant-negative construct, still able to be recruited to the active receptor but impaired in E3 ligase activity, was shown to alter FGFR trafficking, although not by impairing receptor internalization, but rather by affecting its subsequent lysosomal targeting. Although the extracellular FGF8 gradient remained unchanged, the Cbl dominant negative construct was able to cause the accumulation of active endocytosed FGFR in intracellular compartments, resulting in an extended range of FGF-target gene expression and a broader cellular response to gradient. Thus, the investigators were able to conclude that altered endocytic trafficking influenced the way the morphogen was interpreted by the cell (Nowak et al. 2011).
Recent studies are also highlighting the impact of signal compartmentalization in Wnt signaling. This pathway, which is activated when a member of the Wnt family of ligands (Wingless in Drosophila) binds to a receptor of the Frizzled family and to a coreceptor (frequently LPR6), is involved in many developmental processes and its misregulation causes improper fate specification, tumor formation, and early lethality (Cadigan and Nusse 1997). Initial studies, in Drosophila cells and in the whole animal, revealed that interference with endocytic routes (obtained by ablation of dynamin or Rab5) reduced the activity of the pathway (Seto and Bellen 2006). It is to be noted that Wnt signaling is operational in paracrine and autocrine situations, rendering it difficult to understand whether the impact of endocytosis is on the “ligand branch” of the pathway or on the “receptor branch.” Perhaps not surprisingly, the answer seems to be “on both.”
Endocytosis and trafficking play a crucial role in the secretion and pattern distribution of Wnt ligands, thereby regulating their availability (Port and Basler 2010). One critical player in this regard is represented by the multispan integral membrane protein, Wntless (Wls), also known as Evi/Sprinter/GPR177, which is a specific mediator of Wnt ligand secretion and is highly conserved across evolution (Banziger et al. 2006; Bartscherer et al. 2006; Goodman et al. 2006; Fu et al. 2009; Kim et al. 2009). Wls directly binds to Wg (the Drosophila Wnt ligand)—through posttranslational lipidation of the ligand—enabling its transport along the secretory pathway and secretion (Banziger et al. 2006; Bartscherer et al. 2006; Ching and Nusse 2006; Goodman et al. 2006). Endocytosis kicks in with at least two different mechanisms (Koles and Budnik 2012). On the one hand, once exposed on the PM, Wls is continuously internalized and recycled from endosomes to the trans-Golgi network, thereby allowing further cycles of interaction with Wg and release of the ligand (Belenkaya et al. 2008; Franch-Marro et al. 2008; Pan et al. 2008; Port et al. 2008; Yang et al. 2008; Kim et al. 2009; Harterink et al. 2011). On the other, studies in the Drosophila neuromuscular junction revealed that Wg and Wls can be internalized together and then packaged into exosomes that are formed at the level of MVBs. Exosomes are then released in the extracellular space and may facilitate Wg presentation at the postsynaptic site (Korkut et al. 2009, 2013). This mechanism might explain how the hydrophobic secreted Wg ligand could easily traffic in the extracellular space and reach its target site.
Endocytic-mediated segregation of molecules in distinct compartments also regulates Wnt signaling at the level of the “receptor branch.” In unstimulated cells, β-catenin is phoshorylated by glycogen synthase kinase (GSK-3), a signal for its subsequent polyubiquitination and proteasomal degradation. On Wnt signaling, cytosolic β-catenin is instead stabilized and translocates to the nucleus to activate specific transcriptional programs (Moon 2005; Clevers and Nusse 2012). The mechanism of β-catenin stabilization has eluded us until recently, but studies in the Xenopus embryo, now suggest that GSK-3 might be sequestered into the intraluminal vesicles of MVBs, away from its substrates (including β-catenin), after it is internalized from the PM together with a multiprotein signalosome complex (the LPR6-signalosome) containing Frizzled and LPR6 (Taelman et al. 2010). Sequestration requires the action of two ESCRT components, HRS and VPS4, both of which are required for efficient β-catenin target gene transcription in vitro and for Wnt-dependent axis duplication in Xenopus embryos (Taelman et al. 2010). Because GSK-3 phosphorylates many substrates, MVB sequestration might represent a global mechanism promoting Wnt-dependent protein stability.
Chuyên mục: Hỏi Đáp